Rayleigh, Raman, luminescence, fluorescence and phosphorescence emissions from a sample provide an enormous range of information about the sample chemistry and construction. Most biological and organic materials absorb strongly in the deep UV, corresponding to their first electronic state, and emit fluorescence and phosphorescence at longer wavelengths. Luminescence, fluorescence and phosphorescence spectra are independent of excitation wavelength. Asher2 showed that the range of emission wavelengths due to these processes is generally limited to wavelengths above about 260nm. Very few materials fluoresce or phosphorese below this wavelength. Raman spectra, on the contrary, are dependent on the excitation wavelength and are measured in molecular vibration energy terms above (Stokes) or below (anti-Stokes) the excitation wavelength. Therefore, as the excitation wavelength is reduced below the lower limit of fluorescence, there is a fluorescence-background-free region above the excitation wavelength in which to observe the normally weak Raman emissions. Figure 1, below, graphically illustrates this.
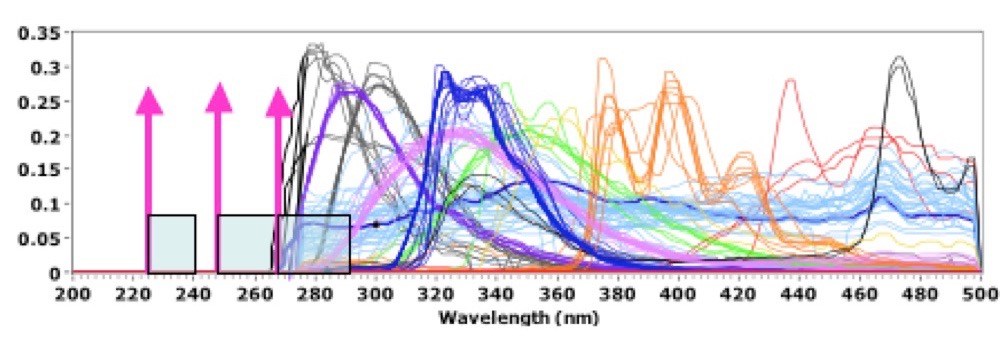
The pale blue band above each of the excitation wavelengths shown in Fig.1 correspond to a 3000 cm-1 Raman shift range. Illustrated is the fact that deep UV Raman excitation at 224nm provides a fluorescence-background-free range for well over 3000 cm-1. Excitation at 248nm is fluorescence-background-free for all but a few material, and only at the largest Raman shifts. Excitation at 266nm occurs directly in the middle of the autofluorescence of a wide range of biological and organic chemical materials. In addition, the water Raman band for a 266nm laser occurs in the middle of biological autofluorescence bands, making it difficult to use these lasers for biological identification using autofluorescence. And excitation at longer wavelength further exacerbates the problem of fluorescence interference, just as the Raman signals themselves are diminished by Rayleigh law and other considerations. Deep UV Raman spectroscopy has five primary advantages over near UV, visible or near-IR Raman spectroscopy summarized as: Rayleigh law, resonance Raman, simplification of spectra, and fluorescence-free, and solar-blind background.
Raman scatter cross section of any material depends as the inverse fourth power on excitation wavelength, called the Rayleigh scattering law. The Raman cross-section of any Raman band is 20X larger at 248nm than at 532nm and 100 times larger than at 785nm. Overlayed on this signal improvement are pre-resonance or resonance effects which can provide additional increase in Raman cross-sections by factors of 5 or 10 up to several million times. Just between excitation at 532nm and 248nm pre-resonance effects for water increase the Raman cross-section about 6X for a total Raman cross-section increase due to Rayleigh and pre-resonance of 120X. The Raman cross-section of water, including both Rayleigh and pre-resonance effects, is 570X between 785nm and 248nm. This means that a 785nm laser requires 570X more power to achieve the same Raman signal as a 248nm laser. The additional signal enhancement is due to pre-resonance of the water molecule, illustrated by the fact that the experimental data most closely follows the Albrecht A-term in which the lowest electronic state dominates the pre-resonance intensity enhancement3. The Albrecht A-term expression gives the following frequency dependence of the Raman cross sections.
σR = A x νo(νo - νR)3[((νe2 + νo2)/ (νe2 - νo2)2) + B]2 Equation 1
where νR = the Raman frequency (cm-1); νo = the laser frequency (cm-1); νe = the frequency of the transition to the excited state (cm-1), and A and B = constants. The parameters A, B and νe are adjusted to fit the curve to the experimental σR versus νo data.
When the difference between the laser excitation frequency, νo, and the frequency of the transition to the excited electronic state, νe, goes to zero, the Raman scatter cross-section in Eq. 1 [(νe2 + νo2)/ (νe2 - νo2)2] goes to infinity. In practice, the cross section goes to a very large value, in the millions, but not infinity. Resonance Raman is especially important for organic and biological molecules since strong absorption typically occurs in the deep UV and resonance enhancements of millions of times have been reported4.
One of the factors limiting the enhancement of Raman scattering when excitation is at or near resonance is absorption of the excitation energy as well as self-absorption of the Raman scattered radiation within the scattering molecules. The Raman signal detected by a sensor is proportional to the Raman cross section, σR, the molecular density of the scattering material, ρ, and the amount of absorbance of the laser penetration into the absorbing material at the laser wavelength, αo, as well as the amount of absorbance of the Raman scattered light reabsorbed by the sample, as described below
D∫ - ( α + α ) r Eq.2. Ramansignal∝σRxρ 10 o R dr Equation2
0 where ρ = molecular density, D = sample thickness, αo = absorbance per unit length at the laser wavelength, and αR = absorbance at the Raman emission wavelength5. When looking for trace levels of contamination where contaminating materials or particles are of the size of 1 μm to 10 μm, this is not expected to be a limiting problem. The third advantage of operating in the deep UV where electronic resonance effects are important is that the Raman spectra are simplified since resonance enhancement occurs only within the Raman bands associated with the electronic transition6. Simplification of the Raman band structure enables easier interpretation of results, which often include complex overlapping band structures for organic and biological materials with normal Raman scattering7,8.
The fourth very important advantage of operating in the deep UV below about 250nm is the absence of background fluorescence in the Raman spectral range. Raman scattering is a far less efficient process than Rayleigh scattering or fluorescence. Typically Raman scattering is 104 to 108 times less efficient than fluorescence. Therefore if any fluorescence process occurs within the target molecules or surrounding materials within the exposure volume of the excitation laser beam, it will overwhelm the weak Raman emissions. For excitation in the visible, the fluorescence efficiency of many materials is over 10,000 to 100,000 times greater than Raman scattering efficiency. Even in the deep UV fluorescence is still at least 500 to 1000 times greater than Raman scattering, unless excitation occurs below about 250nm. Asher2,3,7 showed that organic materials did not fluoresce below a wavelength about 270nm, independent of the excitation wavelength. This was further proven in many subsequent publications such as Nelson (ref. 8), Sparrow6, Wu9, and many others. Therefore, when excitation occurs below about 250nm, a fluorescence-free region extends from the excitation wavelength to over 4000 cm-1 in which to observe Raman spectra. This is not the case for lasers that provide excitation at longer wavelengths. Even excitation at 266nm from a 4th harmonic Nd-YVO4 laser, or equivalent, has most of it’s Raman spectral range overlapped with fluorescence from a wide range of organic and mineral materials as illustrated in Fig. 1.
The final advantage of deep UV Raman and fluorescence is elimination of solar background. Solar background is essentially eliminated below about 300 nm. But the addition of the gated detection described later also serves to reduce the effect of any solar background.
REFERENCES
1 Relman, D.A., “Detection and Identification of Previously Unrecognized Microbial Pathogens”, Emerging Infectious Diseases, Vol.4, No. 3, July-Sept. 1998.
2 S.A.Asher, C.R. Johnson, “Raman Spectroscopy of a Coal Liquid Shows That Fluorescence Interference Is Minimized with Ultraviolet Excitation”, Science, 225, 311-313, 20 July 1984.
3 Asher, S.A. and C.R. Johnson, “UV Resonance Raman Excitation Profile Through the 1B2 State of Benzene”, J. Phys. Chem. Vol89, 1985, pp. 1375-1379.
4 Li, B., and A.B. Myers, “Absolute Raman Cross Sections for Cyclohexane, Acetonitrile, and Water in the Far Ultraviolet”, J. Phys. Chem. Vol94, 1990, pp. 4051-4054.
5 Christesen, S., J.M. Lochner, A.M. Hyre, and Darren K. Emge, “UV Raman Spectra and Cross Sections of Chemical Agents”, SPIE, Vol. 6218, 621809, April, 2006.
6 Sparrow, M.C., J.F. Jackovitz, C.H. Munro, W.F. Hug, and S.A. Asher, “A New 224nm Hollow Cathode UV Laser Raman Spectrometer”, App. Spect., Vol. 55, No. 1, Jan 2001.
7 Asher, S.A., “UV Raman Spectroscopy”, Anal. Chem., Vol. 65, 1993, p. 59 and 210.
8 Nelson, W.H., R. Manoharan and J.F. Sperry, “UV Resonance Raman Studies of Bacteria”, App. Spect. Reviews, 27 (1), pp. 67-124, 1992
9 Wu, M., M.Ray, K.H.Fung, M.W. Ruckman, D. Harder, and A.J. Sedlacek, “Stand-off Detection of Chemicals by UV Raman Spectroscopy”, App. Spect., Vol.54, No.6, 2000, pp. 800-806.
10 W.H.Hug, R.Bhartia, A.Tsapin, A.L.Lane, P.G.Conrad, K. Sigapati, and R.D. Reid, “Status of Miniature Integrated UV Resonance Fluorescence and Raman Sensors for Detection and Identification of Biochemical Warfare Agents”, Proc. SPIE, Vol. 5994, p5884J1-12, Boston, MA. Oct. 2005.
Get In Touch
Keep informed about the latest deep UV developments at Photon Systems by joining our mailing list.
ADDRESS
1512 Industrial Park St. Covina, CA 91722-3417
PHONE
626 967-6431